Integrated Quantum Photonics
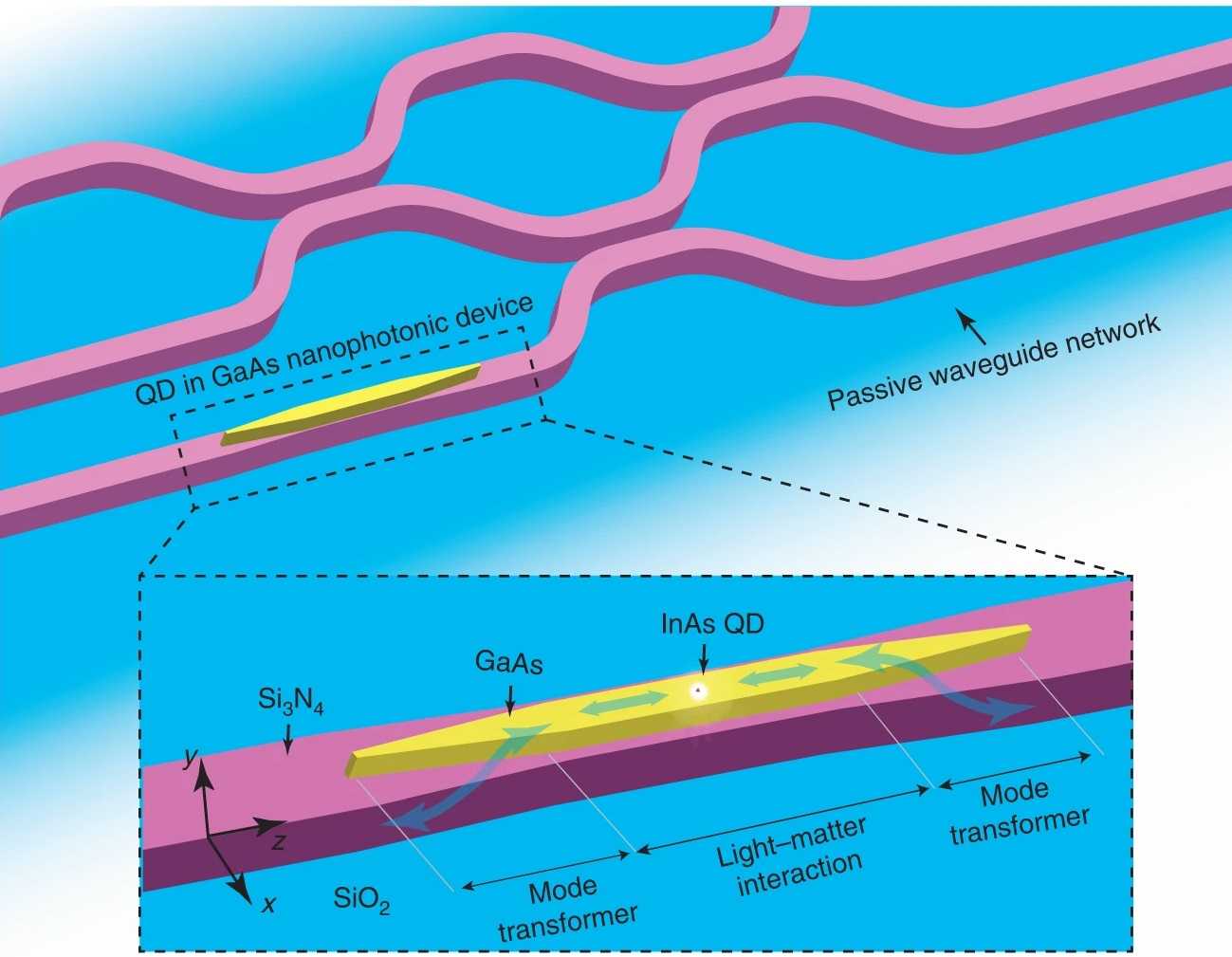
Schematic illustrating the heterogeneous integration of a single-photon source based on a InAs/GaAs quantum dot device that is heterogeneousl integrated with a silicon nitride photonic integrated circuit (Davanco et al, Nature Communications, 2017).
Motivation:
Photonics is likely to play an important role in future quantum technologies for computing, communications, sensing, and metrology.
In computing, the scalable manufacturing of complex photonic networks on a chip may enable both specialized, fit-to-purpose quantum simulators and more general quantum computers (e.g., based on linear optics quantum computing architectures). Photonic quantum interfaces that, for example, realize efficient and low-noise transduction of quantum states of light to and from the telecommunications band may be needed to enable distributed quantum computation based on superconducting quantum circuit or trapped ion quantum nodes that natively operate in the microwave and visible/UV, respectively.
In communications, quantum states of light such as single photon Fock states, entangled photon pairs, and photonic cluster states are carriers of quantum information and in the optical domain, are uniquely suited for transmission without degradation from thermal background photons (in contrast to operation in the microwave domain). The aforementioned quantum transducers may play a key role in enabling quantum repeaters based on quantum memories that operate in the visible but are linked by photons operating in the telecommunications band.
In sensing and metrology, laser light provides low-noise and ultra-high-bandwidth readout of physical processes. The exquisite optical coherence made possible by, for example, stabilizing a laser source to a high-Q atomic transition forms the basis of clocks whose accuracy and precision can enable fundamental tests of physical theories. Quantum states of light, most notably squeezed states, provide an additional resource that can avoid back-action and realize measurements below the standard quantum limit.
In all of the above areas, integrated photonics offers the ability to realize core functionalities in a platform that is suitable for scalable manufacturing and deployment outside of a laboratory.
Summary of our work:
We realize physical functionalities of relevance to quantum information science by using nanoscale fabrication technology to create photonic geometries that enhance light-matter interactions relative to conventional systems. The specifc light-matter interaction that we try to enhance depends on the application. A selected list of representative publications is included below, with the full list available on the Publications page.
Quantum light sources:
Quantum states of light can be created using both single quantum emitters and parametric nonlinear processes, but the former is better suited for creating high-brightness, on-demand sources while the latter is better suited for creating tailored frequency domain entanglement in situations for which heralding can be used. Taking this a step further, for working with single quantum emitters, we typically consider structures that enhance the effective dipole coupling to the electromagnetic field, ideally to the extent that only a single mode of the electromagnetic field shows appreciable interaction, with that mode being one that can be well-coupled to a useful excitation/detection channel, such as the mode of an optical fiber. For sources based on nonlinear optics, we consider geometries that enable effective phase and frequency-matching for all of the spectrally distinct electromagnetic modes involved, and try to understand how to efficiently in and out-couple light at these different frequencies.
The model quantum emitter system we typically use is a self-assembled III-V semiconductor quantum dot grown via molecular beam epitaxy. We have been fortunate to work with quantum dot material provided by KIST, the University of Rochester/Ottawa, TU Berlin, JKU Linz, and the University of Wurzburg. Our research has involved efforts to create suitably engineered photonic geometries to enhance the quantum dot radiative emission into a targeted mode that can be well-collected (Davanco et al, Appl. Phys. Lett., 2011), attempts to understand the influence of nanofabrication on quantum dot blinking and spectral diffusion (Davanco et al, Phys. Rev. B, 2014 and Liu et al, Phys. Rev. Appl, 2019), the heterogeneous integration of quantum dot containing devices with low-loss photonic circuits (Davanco et al, Nat. Comm. 2017), and optical imaging methods to spatially locate quantum dots that typically appear randomly in the plane of the sample growth with high precision and accuracy (Sapienza et al, Nat. Comm, 2015), so that subsequent device fabrication is done in an optimal way (i.e., the quantum dot is optimally located with respect to the optical field). We are continuing to develop these quantum-dot-based sources for both on-chip and off-chip applications, ideally in a way that enables simultaneously bright, pure, and indistinguishable photons to be generated into a desirable photonic channel, which may be an on-chip waveguide or a single-mode optical fiber, for example.
Our work in quantum sources based on nonlinear nanophotonics has included the creation of heralded telecommunications-band single-photon sources in silicon (Davanco et al, Appl. Phys. Lett, 2012) and narrow-linewidth, visible-telecom entangled photon pair sources in silicon nitride (Lu et al, Nat. Phys., 2019). Both of these sources utilize spontaneous four-wave mixing, and this work is closely coupled to our research on quantum frequency conversion and more generally on nonlinear nanophotonics, as much of the fundamental device design and underlying physics is the same. We are continuing to develop the visibel-telecom photon pair source as a means to interface distant quantum memories via entanglement swapping.
Our research on both types of sources has benefitted from essential collaborations within NIST (both Gaithersburg and Boulder campuse), University of Rochester, TU Berlin, University of Wurzburg, Politecnico di Milano, University of Southampton, UC San Diego, IBM, Photon Spot, and Sun-Yat Sen University.
Quantum frequency converters:
Quantum frequency conversion (QFC) is a necessary ingredient in quantum systems that consist of frequency-disparate photonic quantum technologies. Most obviously this could include quantum nodes made of different physical technologies (e.g., a quantum dot and a trapped atom), but it may also include visible wavelength quantum nodes and long-distance fiber optic links (where the latter perform significantly better at telecommunications wavelengths), solid-state quantum emitters that suffer from inhomogeneity due to variations in their size, shape, composition, or surrounding environment, or situations in which more optimal photon detectors are available at wavelengths that differ from the emission/propagation wavelengths.
Our early work (2010-2013) on QFC focused on extending the well-developed classical frequency conversion technology based on centimeter-scale periodically-poled lithium niobate (PPLN) waveguides to the domain of single photons produced by a quantum dot. In practice, the difference between a quantum frequency converter and a classical frequency converter comes down to added noise. For QFC, we want to shift the frequency of the quantum state with high efficiency and without the introduction of spurious nosie photons into that target frequency channel. As our input may be a single-photon source, the level of added noise that is permissible is generally far less than what might be needed in most classical applications. For QFC based on three-wave mixing (sum-frequency or difference-frequency generation), this typically involves a choice of pump wavelength that limits noise due to processes like Raman scattering and parametric fluorescence (of course, this pump wavelength must be consistent with phase-matching for the QFC device). Using PPLN waeguides, we demonstrated QFC of QD photons from the telecom O-band (1300 nm) to the visible (700 nm) (Rakher et al, Nat. Phot., 2010), and ultralow noise QFC from 980 nm to 600 nm (Ates et al, Phys. Rev. Lett., 2012), as well as the ability to simultaneously modulate the single photon wavepackets (Rakher et al, Phys. Rev. Lett., 2011) and to make spectrally distinguishable photons indistinguishable (Ates et al, Phys. Rev. Lett., 2012). Preservation of photon antibunching, photon coherence, and quantum interference were shown in these early works.
For the last few years, we have focused on developing new QFC devices based on silicon nanophotonics. In comparison to PPLN waveguides, this is a much newer technology, with the promise of realizing significantly more compact, low power, and customizable devices that can be integrated with other quantum photonic technologies within a common platform and which can be created using scalable manufacturing technology that is available from commercial nanofabrication foundries. However, this new technology is also far less mature, so that much work needs to be done to establish that the frequency converters can be efficient and low noise. As silicon-based materials lack a second-order nonlinearity in bulk, sum- and difference-frequency generation via three-wave mixing as in PPLN has not been our baseline approach. Instead, we use four-wave mixing, and in particular, the four-wave mixing Bragg scattering process, whose Hamiltonian is equivalent to that of the three-wave mixing QFC devices and has the form of an active beamsplitter. After some initial work based on silicon nitride waveguides (Agha et al, Optics Letters, 2013 and Agha et al, Optics Express, 2014), where we shoed phase-matched operation with conversion efficiencies at the few percent level using high-power (Watt-level) pumps, we demonstrated that this process can be efficient and low-noise in silicon nitride microresonators, where the pump power required is in the ten milliWatt range (due to resonant enhancement) and efficiencies as high as 60 % can be realized (Li et al, Nat. Phot., 2016). Most recently, we have used these frequency conversion devices to demonstrate QFC of single photons from a quantum dot single-photon source (Singh et al, Optica, 2019), as well as the tunable quantum interference of single photons that were initially spectrally distinct (Li et al, Phys. Rev. Appl., 2019). We are continuing to develop this type of frequency converter, in terms of further improvement in conversion efficiency, understanding and suppression of noise sources, and connecting different wavelengths of relevance to the photonic quantum information science community. We are also considering how nanophotonics can be used to bridge especially large and important spectral gaps, for example, between the visible and telecommunications bands, where added noise during the QFC process is especially concerning when using typical second-order sum/difference frequency generation and four-wave mixing Bragg scattering. We have recently proposed third-order sum and difference frequency generation as a compelling approach for tackling this challenging problem (Lu et al, Optics Letters, 2021).
Finally, we are also developing QFC devices that connect the microwave and optical domains, though these systems currently lack the efficiency to work with single photons. This work is described further in the 'Nanoscale electro-optomechanical transducers' Research Area.
Our research on QFC has benefitted from essential collaboations within NIST (both Gaithersburg and Boulder campuse), University of Rochester, Photon Spot, University of Wurzburg, and Sun-Yat Sen University.
Quantum sensing/metrology:
Many of the techniques we are developing for quantum light source (e.g., efficient photon collection) also have relevance for quantum sensors, such as those based on spin-resolved fluorescence detection from single quantum emitters (most notably, vacancy centers in diamond). Other work we do on cavity optomechanical systems ('Nanoscale electro-optomechanical transducers' Research Area) also has relevance to quantum sensing. For example, our devices have recently been used in experiments aimed at creating thermometers in which the measured temperature, which is related to the thermal Brownian motion of the mechanical resonator, is calibrated to photon shot noise, which is coupled to mechanical motion via radiation pressure (Purdy et al, Science, 2017). We continue to work with NIST/University of Maryland and the University of Pittsburgh to develop devices of relevance to such metrology goals.